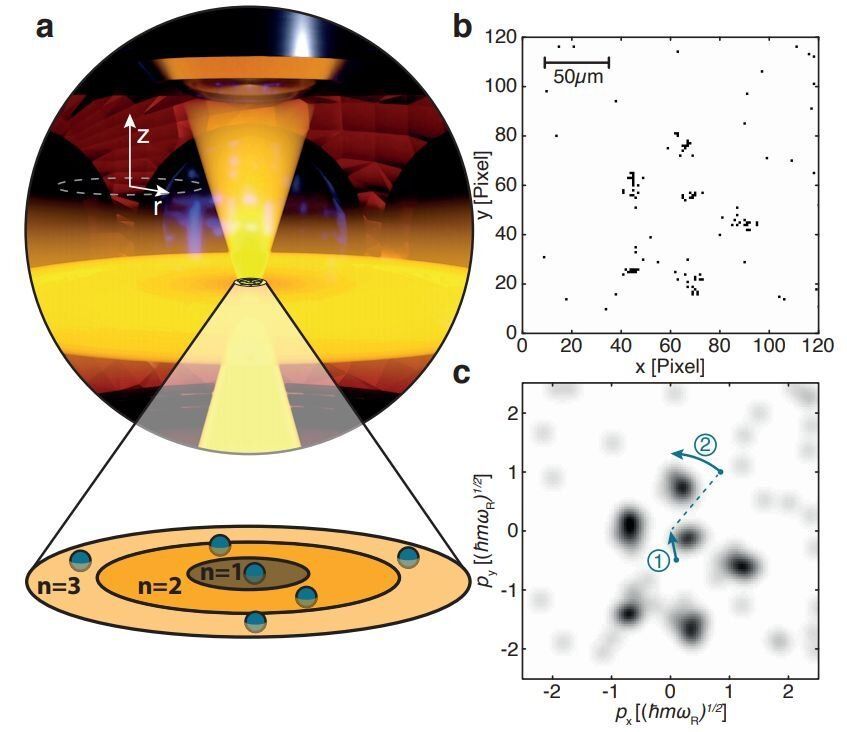
A team of researchers at Heidelberg University has succeeded in building an apparatus that allowed them to observe Pauli crystals for the first time. They have written a paper describing their efforts and have uploaded it to the arXiv preprint server.
The Pauli exclusion principle is quite simple: It asserts that no two fermions can have the same quantum number. But as with many principles in physics, this simple assertion has had a profound impact on quantum mechanics. Looking more closely at the principle reveals that it also suggests that no two fermions can occupy the same quantum state. And that means that electrons must have different orbits around a nucleus, and by extension, it explains why atoms have volume. This understanding of the self-ordering of fermions has led to other findings—for instance, that they should form crystals with a specific geometry, which are now known as Pauli crystals. When this observation was first made, it was understood that such crystal formation could only happen under unique circumstances. In this new effort, the researchers have resolved the circumstances, and in so doing, have built an apparatus that allowed them to observe Pauli crystals for the first time.
The work involved a setup that included lasers that were able to trap a cloud of lithium-6 atoms supercooled to their lower energy state, forcing them to adhere to the exclusion principle, in a one-atom thick flat layer. The team then used a technique that allowed them to photograph the atoms when they were in a particular given state—and only those atoms. They then used the camera to take 20,000 pictures, but used only those that showed the right number of atoms—-indicating that they were adhering to the Pauli exclusion principle. Next, the team processed the remaining images to remove the impact of overall momentum in the atom cloud, rotated them properly, and then superimposed thousands of them, revealing the momentum distribution of the individual atoms —that was the point at which crystal structures began to emerge in the photographs, just as was predicted by theory. The researchers note that their technique could also be used to study other effects related to fermion-based gases.